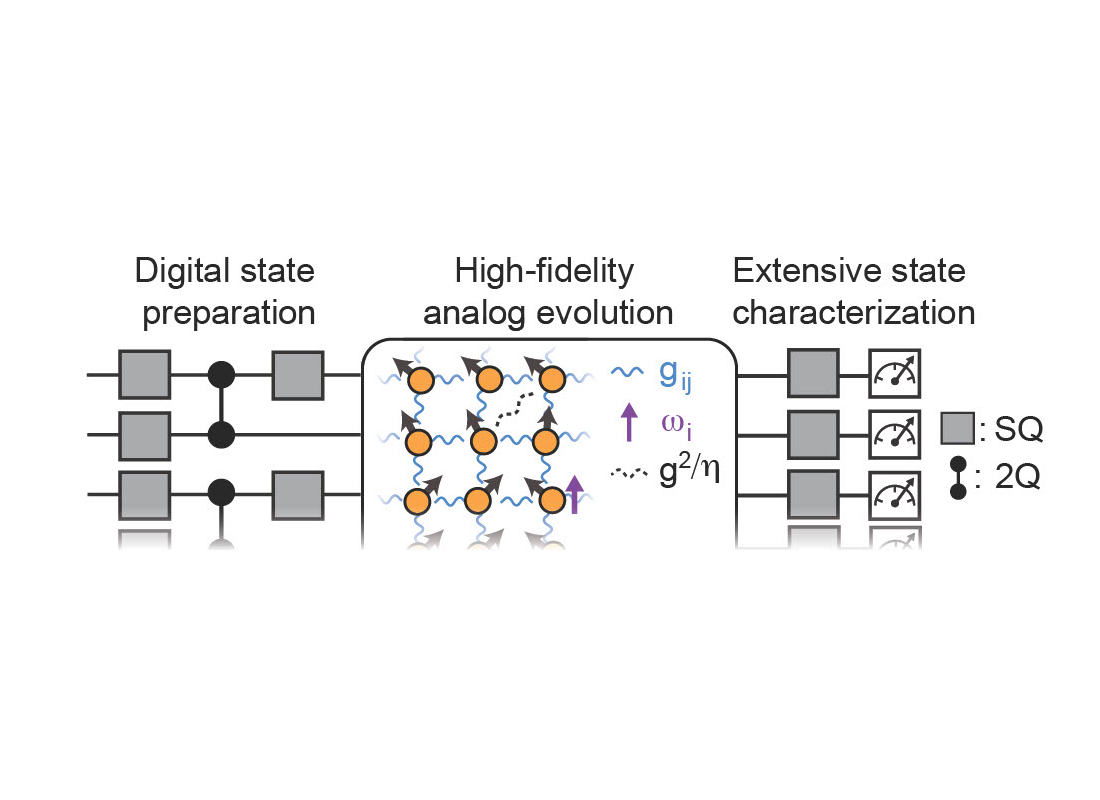
A new hybrid platform for quantum simulation of magnetism
April 21, 2025
Trond I. Andersen, Senior Research Scientist, and Nikita Astrakhantsev, Research Scientist, Google Quantum AI
We have developed a novel hybrid approach to quantum simulation, enabling scientific discoveries and opening new possibilities for beyond-classical applications.
Quick links
A primary goal in quantum computing is quantum simulation of physical systems that are too complex to be studied on even the world’s best classical computers. To achieve this, two features are of particular importance: flexibility and speed. First, a quantum computer needs to be flexible enough to target a wide range of relevant problems. Second, since a key challenge in quantum simulation is the effect of system noise during the simulation, the simulated dynamics need to be sufficiently fast in order to reach interesting quantum states before the noise becomes dominant.
In our recent work, “Thermalization and criticality on an analogue–digital quantum simulator”, published in Nature, we demonstrate how a hybrid approach that combines analog and digital quantum simulation using a 69 qubit device can achieve both flexibility and speed. Leveraging this new technique, we studied a paradigmatic model of quantum magnetism and discovered a surprising exception to a widely used physics theory.
The power of analog–digital quantum simulation
To fully understand the efficacy of this hybrid approach, we need to consider its two modes of operation individually. As an example, consider the simulation of three particles interacting with each other. In digital quantum simulation, the simulated evolution of the three particles is divided into many smaller operations (logical gates) in which individual pairs of particles interact. We simulate this by turning on couplers between corresponding pairs of qubits. Constructing the digital simulation from these smaller building blocks provides immense flexibility, but runs slowly since any given qubit only interacts with one of its four neighbors at a time.
An analog quantum simulator, on the other hand, directly simulates the continuous dynamics of these particle interactions by activating all the couplings between the qubits in parallel. This is more similar to real-world dynamics; after all, real particles do not interact sequentially in a pairwise manner, but rather all at the same time. Crucially, keeping all the couplings active enables faster dynamics. More specifically, it enables a faster growth of quantum entanglement, the key resource of quantum computing. Combining the two approaches brings together the flexibility of digital methods with the fast entanglement growth of analog simulation.
High-precision analog dynamics with new calibration scheme
The key obstacle to realizing the hybrid approach on superconducting quantum hardware is calibrating the analog component. While a digital simulation mainly requires the calibration of each constituent operation, similar to performing a series of well-rehearsed dance moves, analog simulation is like performing all the dance moves at the same time. This is difficult because the effects of the couplers interfere with each other, so that the total result is different from the sum of each coupling acting on its own.
We were able to solve this challenge by developing a new calibration scheme that involves a set of carefully chosen calibration experiments combined with extremely precise modeling of the underlying hardware. This allows us to perform the dance very precisely with comparable accuracy to what we achieve in digital mode.
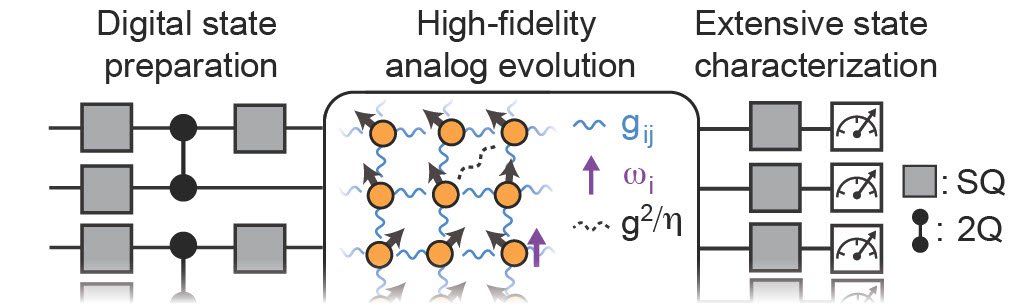
Hybrid analog-digital quantum simulation. In the hybrid approach, we use digital gates to prepare the initial state (left), then evolve to interesting quantum states with high-accuracy analog dynamics (middle), and last, switch back to digital mode for characterization of the final state (right).
We benchmarked the performance of our new analog calibration technique using an experiment known as random circuit sampling. Specifically, we used the analog evolution to reach a chaotic quantum state, i.e., a superposition of all the possible sequences of bits that contain equally many 1s as 0s. For instance, if we had only four qubits, there would be some probability of finding the system in the 1010-state, another probability of the 1100-state, and so on. Then we measured the distribution of these probabilities by repeatedly reading out the state of the system and compared it to our theoretical prediction.
This study revealed two key insights: First, it showed that our analog simulator reaches the chaotic state very quickly, allowing us to access very complex (i.e., highly entangled) quantum states with only very weak noise effects. Second, it showed that the measured distribution agreed very well with the theoretically predicted one, indicating that our calibration enables analog simulation with very high precision. In particular, we determined that the chance of making an error was only 1/1000 each time a 1 moves from one qubit to another. The combination of the fast entanglement growth and high precision makes it challenging to perform equally precise simulations on a classical computer. In fact, we estimate that it would take more than a million years to simulate this benchmarking experiment with the same level of accuracy on Frontier, the world’s fastest supercomputer at the time of our experiment.
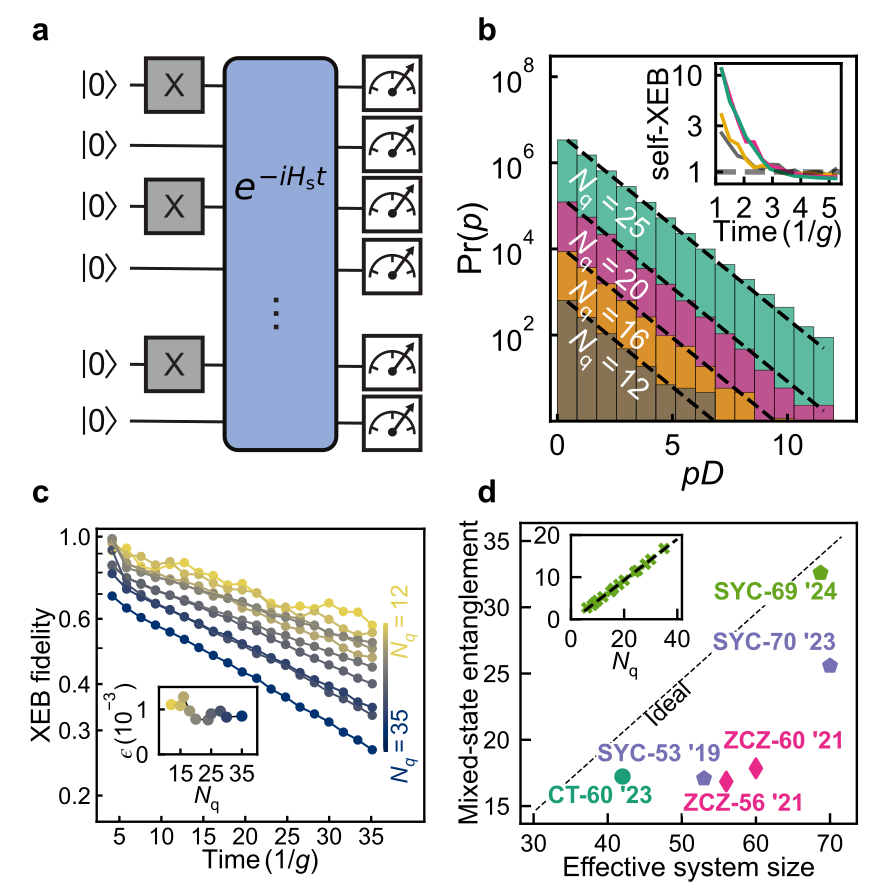
High-accuracy analog performance beyond the reach of classical computers. a) Diagram of the circuit we used to benchmark the performance of our analog simulations using random circuit sampling. b) The measured distribution of bit sequences (see text) indicates that our system reached a highly entangled chaotic state much faster than in digital operation, thus allowing for less accumulation of noise. c) The accuracy (XEB fidelity) decays very slowly over the duration of the simulation, indicating a low error of only 0.1% each time a 1 moves between qubits (inset). d) This makes our platform (SYC-69 ‘24: Google’s Sycamore) very competitive with others, including cold atoms (CT-60 ‘23) and digitally operated superconducting qubits (SYC-70 ‘23 and SYC-53 ‘19: Sycamore; ZCZ-60 ‘21 and ZCZ-56 ‘21: Zuchongzhi processor) in terms of reaching complex and pure quantum states.
Simulating a quantum magnet in the hybrid approach
Having demonstrated accurate analog evolution, we then combined it with our more traditional specialty, high-precision digital gates, to study new physical phenomena. Leveraging our hybrid approach, we simulated a magnet, the behavior of which is very closely mimicked by the natural dynamics on our hardware. Each qubit can be thought of as a magnetic spin — think a little bar magnet — that interacts with its neighbors. We wanted to study what happens to the magnet when the interactions are turned on at varying rates, both because it is an interesting physics question that has attracted substantial attention in the field, and because it can improve our understanding of important techniques in quantum computing, such as quantum annealing.
To simulate this, we first used digital gates to initialize the qubits in an alternating pattern of 1s and 0s, representing spins pointing up and down, respectively. Then we ramped up the analog interactions between the spins at varying rates before switching back to digital mode for measurements. Intuitively, if the interactions are turned on very quickly, the magnetic spins are expected to not have time to react and remain stuck in their initial positions. If turned on slowly, on the other hand, they pull and twist on each other, as bar magnets do, and start pointing in the same direction. Indeed, we found that when the analog couplings were turned on very slowly, we were able to reach quantum states in which the spins align in the horizontal plane in a strongly correlated way, equivalent to a very low temperature. Importantly, here we are not referring to the temperature of the quantum chip itself (which is also very cold), but rather to that of the simulated magnet.
Interestingly, we reached sufficiently low temperatures to observe a famous phenomenon known as the Kosterlitz-Thouless transition, which is a sudden change in the degree of alignment of the magnetic spins in a material. Conceptually, this is similar to the way water molecules suddenly align when they freeze.
Highly correlated, low-temperature quantum states, such as those we observed, are the source of many fundamental puzzles in physics and were previously much less accessible with our purely digital scheme. Moreover, the hybrid approach allowed us to probe the transition in a versatile way, including the observation of several characteristic behaviors of the Kosterlitz-Thouless transition, which would not be possible in a purely analog simulation.
New discovery: Breakdown of a famous physics prediction
While the findings described above agreed well with the theories of Kosterlitz and Thouless, we also observed phenomena that did not match our expectations. In particular, there is a famous theory known as the Kibble-Zurek mechanism that provides a simple mathematical prediction for how the alignment of the magnetic spins changes with the rate at which the interactions are ramped up. However, when we varied the ramp rate in our experiment, the measured alignment of the spins was completely different from this prediction! We first thought that there might be something wrong with our calibration, but after performing additional tests, we realized that this was not a fluke, but rather a new physical insight — the Kibble-Zurek mechanism does not hold as universally as previously believed. While the Kibble-Zurek theory predicts that the spins stop aligning at a point during the ramp and get “stuck” in many small independently aligned patches, instead it turns out that these patches can in fact merge into each other. This new insight sheds light on preparing low-temperature states, which is a central task in quantum simulations.
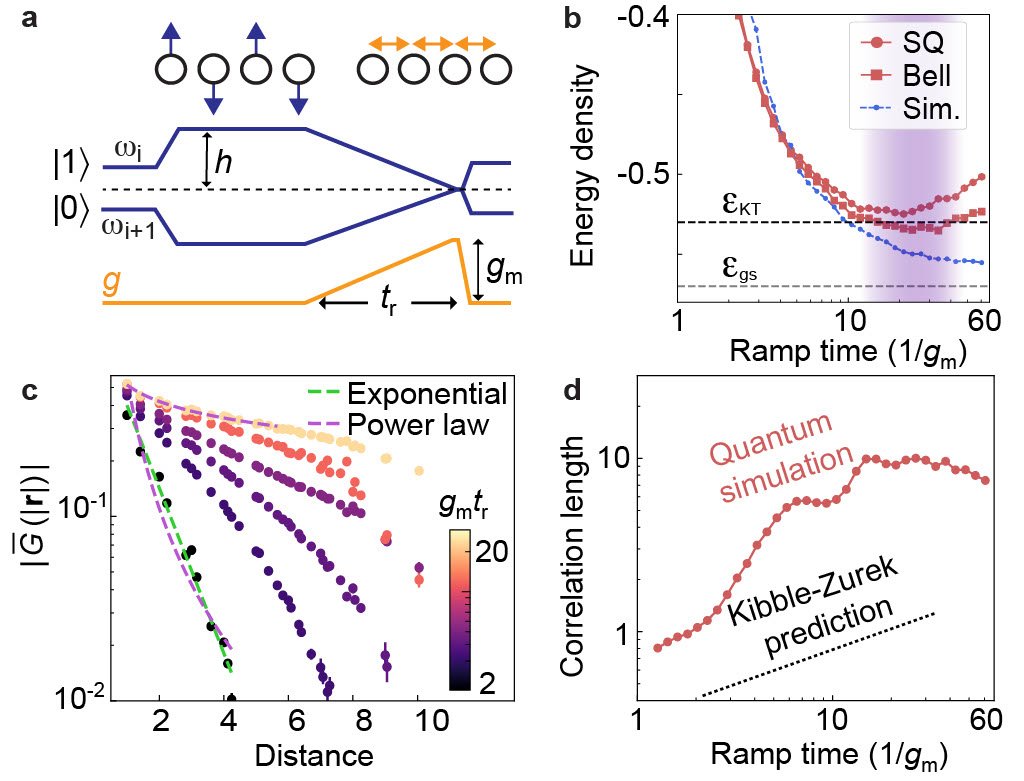
New scientific discovery in simulations of a quantum magnet. a) We prepared the qubits in an alternating pattern of 1s and 0s, representing magnetic spins pointing up and down, and then turned on the interactions (yellow) over a varying ramp time. b) As the ramp time is increased, the energy (i.e., temperature) of the simulated magnet decreases and goes below the expected Kosterlitz-Thouless transition point (dashed horizontal line, εKT). c) The degree of alignment between spins decays with the distance between them. When the ramp is performed slowly (lighter curves), the spins become more aligned and over longer distances. For the slowest ramps, we observe a transition from exponential decay to power-law decay, a signature of the Kosterlitz-Thouless transition. d) Plotting the characteristic length over which spins are aligned (correlation length) versus the ramp time reveals that the Kibble-Zurek prediction (black dashed line) breaks down in our system.
Three-experiments-in-one with the hybrid approach
We continued to push the capabilities of our hybrid approach one step further to access an even broader class of simulations. While the previous experiments used digital gates on single qubits to prepare 1s and 0s, we next introduced two-qubit gates to allow further customization of the properties and spatial structure of the initial state.
To demonstrate the versatility of this approach, we showed that three different initial states each enable different experiments. In the first, we prepared many local patches of low temperature and studied how they merged into each other. In the next, we prepared a hot region next to a cold one and simulated how the two halves equilibrated, a situation similar to that of cold milk being poured into a cup of hot coffee. Finally, we prepared a state that had a flowing current of spins, and studied how the current slowed down over time. This demonstrates that flexible preparation of the initial state is very effective for accessing a wide range of relevant physical phenomena.
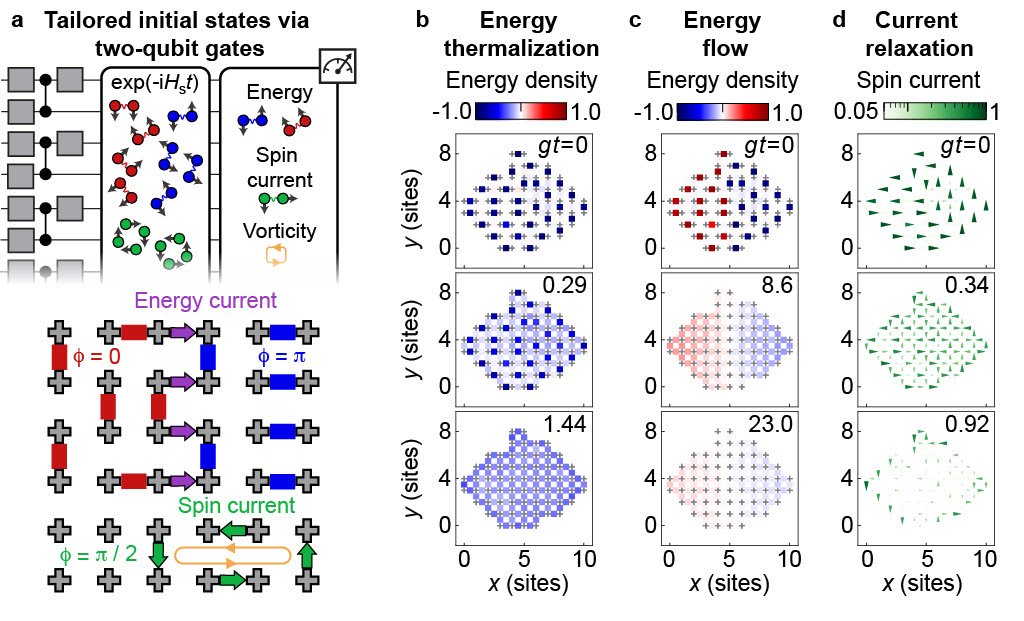
Three-in-one-experiment with customizable initial states. a) Applying two-qubit digital gates at the beginning of the quantum circuit (top) allows us to tailor the properties of the initial state, including the energy landscape and spin currents (bottom). b–d) Preparing different initial states gives access to entirely different dynamics, here shown as snapshots in time (top to bottom). b) Energy redistribution after preparing initial local patches of low energy. c) Energy flow from a hot (red) to a cold (blue) area. d) The relaxation of initial spin currents (green arrows).
Conclusion
We developed a new approach to quantum simulation that combines the best of the analog and digital worlds. Largely enabled by a new high-precision calibration scheme, this hybrid method allowed us to make a scientific discovery and push the limits of what is possible in current quantum simulation experiments. We believe that this is only the beginning of what we will see from this new hybrid approach, as its flexibility and accuracy opens up entirely new directions for using quantum computers to make discoveries that are out of reach for today’s best classical computers. This work was done using Google’s Sycamore quantum chip, and we are thrilled to now also start using this approach on Google’s new chip, Willow, to explore further applications in magnetism and beyond.
-
Labels:
- Quantum